Exploring Undergraduate Biochemistry Students’ Gesture Production Through an Embodied Framework
Abstract
Interpreting three-dimensional models of biological macromolecules is a key skill in biochemistry, closely tied to students’ visuospatial abilities. As students interact with these models and explain biochemical concepts, they often use gesture to complement verbal descriptions. Here, we utilize an embodied cognition-based approach to characterize undergraduate students’ gesture production as they described and interpreted an augmented reality (AR) model of potassium channel structure and function. Our analysis uncovered two emergent patterns of gesture production employed by students, as well as common sets of gestures linked across categories of biochemistry content. Additionally, we present three cases that highlight changes in gesture production following interaction with a 3D AR visualization. Together, these observations highlight the importance of attending to gesture in learner-centered pedagogies in undergraduate biochemistry education.
INTRODUCTION
In biochemistry, many learning goals are related to visuospatial reasoning and focus on the production, modification, evaluation, and interpretation of representations (Voet et al., 2003; Tibell and Rundgren, 2010; Schönborn and Anderson, 2010; Tansey et al., 2013; Loertscher et al., 2014). Engaging with and interpreting models of macromolecular structures in three-dimensional (3D) space are central activities that are closely tied to conceptual development of structure-function relationships within and between macromolecules (Zimmerman, 2003; Tibell and Rundgren, 2010; Tansey et al., 2013). Students’ visuospatial skills, encompassing “skill[s] in representing, transforming, generating, and recalling symbolic, nonlinguistic information”, play an important role in their participation in learning activities to develop a strong understanding of macromolecular structure in 3D space. (Linn and Petersen, 1985, p.1482; Oliver-Hoyo and Babilonia-Rosa, 2017).
Students often learn about molecular structures using external representations that are created by various visualization tools. There is an extensive body of literature documenting the use of digital macromolecular visualization technologies to introduce virtual 3D external representations of macromolecular structure as classroom intervention strategies to increase visuospatial skills, content retention, and confidence in ability to visualize 3D protein structure in undergraduate students (Noriega and Vazquez, 2000; White et al., 2002, 2010; Jaswal et al., 2013; Terrell and Listenberger, 2017; Sung et al., 2020). Within this landscape of existing technologies for engaging with virtual 3D models of macromolecular structure, augmented reality (AR) represents an emerging pedagogical tool by which students and instructors can easily engage with virtual 3D representations of macromolecular structure (Argüello and Dempski, 2020; Peterson et al., 2020; Sung et al., 2020; Rodríguez et al., 2021; Machado et al., 2022). Understanding how students engage with external representations, especially those that utilize new technologies such as AR models, can benefit from an analysis of how students describe the macromolecular structures that these models were designed to represent. To gain insight into students’ descriptions of macromolecular structures, biochemistry education researchers have primarily relied on analyzing students’ verbal expressions and written responses (Rundgren et al., 2012; Harle and Towns, 2013; Barak and Hussein-Farraj, 2013). For example, Harle and Towns (2013) used students’ written responses and drawings to assess their understanding of secondary and tertiary protein structures.
In recent years, developments in cognitive science directed researchers towards exploring the embodied ways in which learners may develop their ideas about visuospatial relationships (Stieff et al., 2016). The inclusion of embodied expressions of thought, for example, gestures and other bodily movements, can complement the traditional focus on verbal expression and provide a more nuanced analysis of students’ behavior (Stieff et al., 2016). Although embodied cognition has been gaining traction in mathematics and science education, the adoption of an embodied perspective remains rare in biochemistry education research, particularly in research on students’ expressions about protein structure and function. The current study contributes to existing literature by adopting an embodied cognition framework to investigate the various ways in which students utilize gestures to describe their proposed mechanistic model of how the potassium channel selectively transport potassium ions. In this article, we will present insights from analyzing the gestures produced by undergraduate biochemistry students as they described the mechanism of the potassium channel.
BACKGROUND
Embodied Cognition and Gesture
The central argument of the embodied cognition perspective relates to “the idea that the body or the body’s interactions with the environment constitute or contribute to cognition” (Shapiro and Spaulding, 2021, para. 1). This means that sensory and motor experiences are pivotal for reasoning, making meaning, and learning (Stolz, 2015; Johnson, 2017; Varela, 2017). For example, when a person reads text about objects and/or action, for example, a nail being hammered, their previous sensory and motor experiences with those objects and/or actions form the basis for how they would attach meaning to the text, sometimes leading them to make implicit inferences, for example, the nail is pointing downwards (Bergen, 2012). Because its emergence, embodied cognition has been utilized in cognitive and educational research in various ways that gave the term different meanings. Kersting et al. (2021) reviewed science education literature and categorized four senses of embodiment that conceptualize the body in physical, phenomenological, ecological, and interactionist terms. These four senses of embodiment afford different focuses and complement each other in the investigation of the role of the body in science learning. The physical sense of embodiment views the body as a biological structure, where the understanding of scientific concepts is grounded in our bodies (Niebert et al., 2012; Bruun and Christiansen, 2016). The phenomenological sense of embodiment views the body as lived experiential structure, where the development of practical scientific knowledge is grounded in our lived experiences (Hardahl et al., 2019; Nikolopoulos and Pardalaki, 2020). The ecological sense of embodiment focuses on the codependence between body, mind, and world, where the processes of student-environment interactions, such as interactions with technology, are given more attention (Bernhard, 2010; Gallagher and Lindgren, 2015). Lastly, the interactionist sense of embodiment focuses on the sociocultural interactions between bodies, where the collaborative, communicative, and socially situated nature of science is recognized as foundational for learning (Danish et al., 2020). The coordination of these four senses of embodiment can produce a rich and complex analysis of students as embodied agents. Through the lens of embodied cognition, physical experiences that come from moving a human body form the basis for cognition (Kersting et al., 2021).
Adding to the complexity of how embodiment is defined, some scholars have also pointed out that the implication of an embodied perspective on cognition could be much more radical: if cognition is no longer isolated in the brain, then the notion of mental states cannot exist (Wilson and Golonka, 2013). Adopting an embodied perspective on cognition is not merely acknowledging the effect of bodily experiences on an otherwise disembodied cognitive system, but fundamentally changing how we conceptualize cognition (Wilson and Golonka, 2013). If resources for cognition are distributed across brain, body, and environment, it follows that we should understand our knowledge and our means of arriving at knowledge in terms of the relationships between them (Hutto and McGivern, 2015; Jensen and Greve, 2019). Although Wilson and Golonka (2013) advocated for a “replacement style” research from an embodied perspective that moves away from the standard assumptions of cognitive psychology that separate brain, body, and environment and take into account brain-body-environment cognitive systems, they acknowledge that abstract cognitive activities involving the use of language remained difficult to explain without the notion of internal representations.
One strain of research in embodied cognition that investigates the embodied ways in which abstract cognitive activities occur, such as the use of language, focuses on the analysis of gesture (Goldin-Meadow, 1999, 2011; Lapaire, 2011). As a manifestation of embodied cognition, gestures, defined as spontaneous hand movements accompanying speech, can be thought of as consisting of two main categories: iconic gestures and metaphoric gestures (McNeill, 1992; Kendon, 2004; Novack and Goldin-Meadow, 2017). Iconic gestures directly relate to the meaning of accompanying speech while metaphoric gestures depict abstract information (Straube et al., 2010). For example, an arched gesture would be considered iconic if made in conjunction with the sentence, “there is a bridge over the river,” because it depicts a physical structure, while this same gesture would be metaphoric if accompanying the phrase, “the politician builds a bridge to the next topic,” because it illustrates an abstract concept (Straube et al., 2010).
Gesture and STEM Education
In STEM education research, scholars have started to include gestures when analyzing students’ conceptual understanding. For example, in mathematics education research, gestures have been used as data for phenomenographic analysis of interview participants’ understanding of the concept of rate (Herbert and Pierce, 2013). Soto-Johnson and Troup (2014) examined how the types of gestures produced by students enrolled in an undergraduate complex variables course shifted as they worked through online lab tasks. Parrill et al. (2019) reported the frequency of gesturing for undergraduate students when they were describing their understanding of the concept of SD. Gestures have been recognized to be often used to communicate information that is either not contained in speech or to emphasize information in speech (McNeill et al., 1994). For example, gesture has been found to complement, supplement, or contradict students’ verbal descriptions of the concept of rate (Herbert and Pierce, 2013). Herbert and Peirce (2013) observed that students produced the “slope hand” gesture to complement their verbal expression “I think it will go that way” when talking about rate of change. The “slope hand” gesture provided supplemental information to student utterance.
Student-produced gestures can also influence how a student thinks about a problem and reciprocally impact their learning (Goldin-Meadow, 2011; Wakefield and Goldin-Meadow, 2021). For instance, it was observed that adults and children who used gesture while explaining mathematical concepts recalled more concepts than those who did not gesture (Goldin-Meadow et al., 2001). Sjøberg et al. (2023) found that a variety of iconic gestures supported undergraduate biology students’ model-based reasoning when creating drawings related to the mechanisms of medical diagnostic tests. In physics education, researchers found that gestures can provide sensorimotor information that help facilitate the learning of physics in classical mechanics (Scherr, 2008; Bruun and Christiansen, 2016).
Research has shown that speakers tend to produce gestures when their verbal expressions contain spatial information (Alibali, 2005). In the context of biochemistry education, including gesture analysis can be productive when investigating how students describe macromolecular 3D structures. For example, Myers (2008) describes the process by which expert crystallographers sculpt protein models through gesture. These embodied models can take many different forms: pulling one’s arm to the side of their body while representing a conformational change; hands pulsing around invisible objects seen on-screen; or fingers tracing the path of molecular interactions (Myers, 2008). This gesturing was observed to not only function as a way to “give body” to digital models, but as a way to train novice crystallographers as they learn to render complex models and develop biochemical intuition (Myers, 2008, 2015). Ackerman et al. (2008) examined how gestures are used by scientists in a biochemistry lab when formulating scientific theories and argued that gestures are essential resources for shaping theoretical understanding. Gestures were found to be frequently used to reference, modify, and embody other forms of external representations such as models, diagrams, and graphs. With gestures, scientists can package theoretical conjectures into a single semiotic form, expressing spatiodynamic information that could not be conveyed efficiently through other modalities (Ackerman et al., 2008). Therefore, analysis of gestures can provide a more complete description of student expressions by attending to nonverbal expressions.
Gesture has also been shown to relate to spatial thinking by allowing students to physically perform spatial transformations (Goldin-Meadow et al., 2009; Stieff et al., 2016). For example, gestures were found to be used by chemistry students when they communicate change molecular structure, where a hand rotation gesture could be used to represent the shift in a molecular bond angle (Goldin-Meadow et al., 2009; Stieff et al., 2016). Conceptualizing gesture as a form of expression is particularly relevant for undergraduate students studying biochemistry because they represent a group of learners developing the skill to express visuospatial information necessary to answer complex biochemical questions. Taken together, this literature suggests that gestural analysis, namely analysis of factors like gesture frequency, gestural dimensionality, and content conveyed by gestures, can provide valuable insight on students’ descriptions of scientific concepts and how students engage with STEM content, complementing traditional research that privileged verbal expression as the primary focus.
In this study, we engage with the embodied perspectives on cognition and consider how students use cospeech gestures when describing their proposed mechanistic model of the potassium channel. This study situates itself in an ecological sense of embodiment and focus on the interplay between speech and gesture in student expressions. Insights from embodied cognition provided an entry point to expand existing approaches to educational research that traditionally emphasize verbal and written responses as forms of student expression (Hajar, 2021). Analyzing cospeech gesture provides a way to include the embodied external representations produced by students as part of their expression. We focus our analysis on only cospeech gestures and we will use the term “gesture” to refer to only cospeech gestures in this study. To better understand the role of gesture production in how students express their proposed mechanistic model of the potassium channel, we aim to describe the emergent themes that students expressed through producing cospeech gestures, the patterns in which students produce cospeech gestures, and the different types of changes in their gestures following engagement with an AR model. This study addresses the following research questions:
What are the themes of students’ cospeech gesture when proposing a mechanistic model of the potassium channel?
What are the patterns of students’ gesture production when describing their model of the potassium channel?
How do students’ gesture production change after interacting with an AR-based learning tool?
Our results are organized into three sections to reflect each of these research questions. In Part I, we present emergent themes of student utterances that were accompanied by cospeech gestures. In Part II, we deepen our analysis of student expression through cospeech gestures by comparing different patterns of gesture production. Lastly, in Part III, we consider the changes in student gesture production following interaction with an AR model of the potassium channel. We will then connect our findings to the previous literature describing students’ use of gesture in STEM, highlighting that undergraduate biochemistry students produced detail-rich cospeech gestures when describing external representations of protein, with great variation in patterns of student gesture production.
METHODS
Participants and Data Collection
The participants of this study consisted of seven undergraduate students enrolled in an introductory biochemistry course at a small, private liberal arts college in the western United States. The prerequisites for enrollment in the biochemistry course included completion of one semester of organic chemistry and two semesters of general chemistry. The study was reviewed and approved (exemption granted) by the Institutional Review Board.
We collected video and audio recordings of semistructured interviews conducted via Zoom where students were tasked with developing and refining a mechanistic model of the potassium channel to explain the molecular mechanism of how the potassium channel selectively transport potassium ions. The interview task was structured where students first proposed a model for the potassium channel without any external representation presented to them, then engaged with an AR visualization of the potassium channel (via BiochemAR), and lastly revised or added additional information to their proposed model based on their interaction with the AR visualization. Students were first asked to consider a set of functional groups and structures (e.g. C-terminus of an alpha helix, basic amino acid side chains, water molecules, etc.) and determine whether or not they would stabilize or destabilize a K+ ion in the center of a membrane bilayer. Next, interviewees were then given basic information about the potassium channel and asked to propose a model of how the K+ ions traverse through the channel. After proposing an initial model, students were introduced to the AR model via BioChemAR. BiochemAR is a free to download mobile application with a learning module that illustrates the role of amino acids and protein secondary structure in facilitating ion transport in the KcsA potassium channel (PDB ID: 1BL8; Doyle et al., 1998; Sung et al., 2020). Students downloaded the app to a mobile device and were provided with a printed QR code that served as the image marker for the AR model. Students were then asked to describe what they were seeing as they familiarized themselves with the potassium channel AR model. Finally, they were tasked with revisiting and modifying their initial hypothesis based on the AR model. The complete interview protocol can be found in Supplemental Table S1. The audio recordings were transcribed verbatim for further analysis. The video recordings were coded and analyzed used the protocol described below.
Gesture Coding and Analysis
Step 1: Gesture Identification.
In the first step of gesture analysis, our objective is to differentiate all visible hand movements by the interviewee into gestures and nongestures. Nongestures were defined as self-touching (e.g., stroking hair) and object manipulation (e.g., picking up a writing utensil); all other motions were defined as gestures. Gestures were described as a combination of hand shape and motion (in some cases accompanied by additional props such as drawings, paper, and/or pencil). Individual gestures were separated by pauses in motion between distinct hand shapes. Gestures were also aligned with their corresponding utterances in the interview transcript. After a minimum of two researchers independently coded the video data to identify gestures, the researchers discussed to reach consensus and generated a collection of gestures that were agreed by all researchers for further analysis. During the discussion, researchers reached agreements on not only what motions were classified as gestures, but also the start and end of each gesture.
Step 2: Gesture Description.
With a collection of gestures identified in Step 1, in this step of the analysis, the goal was to establish detailed descriptions of gestures for further categorization. The hand shape and motion of the gestures were described from the speaker’s viewpoint. Researchers first generated the descriptions independently, then resolved any disagreement through consensus coding. The labels and descriptions of all student-produced gestures can be found in Supplemental Table S2.
Step 3: Gesture Categorization.
For the third step of our analysis, we categorized student gestures into topics based on the utterances that accompanied each gesture (e.g., gestures accompanying a statement regarding the structure of the potassium channel would be categorized as “channel depiction”). All gestures produced across the seven student interviews were categorized into separate topics based on the accompanying utterance. In this step of analysis, gestures that may have the same hand shape and motion are further differentiated based on the content of the accompanying speech (e.g., a gesture described as “tube” could be further differentiated into “channel depiction” and “channel-ion interaction” categories). The categorization of gestures into their respective topics served to take account of the language that students used and how it was combined with gestures to express meaning. In addition to these topics that expressed through gestures, we also categorized these gestures as either iconic or metaphoric based on their relationship with the speech content. Iconic gestures depicted speech content literally, while metaphorical gestures depicted speech content abstractly. Similar to previous steps of analysis, gesture categorization also started with researchers coding gestures independently into topics and followed by consensus coding. The development of these categories also informed the analysis of content within and among student interviews. Table 3 outlines the topics used to categorize student gestures in this study.
Step 4: Combining Speech and Gesture.
The meanings of iconic and metaphoric gestures were interpreted in Step 4 of our analysis. We particularly focused on the interpretation of iconic and metaphoric gestures because these gesture types often serve to express content knowledge. Gestures that occurred when discussing topics that are not directly related to the task were excluded from the results. The meanings of these gestures were interpreted using both the description and content topic of the gesture. The goal of this step of analysis was to determine the intended meaning of student gestures present in video data. As well, gesture interpretation started with researchers independently interpret gestures and followed by consensus coding to reach agreement on the interpretations.
Step 5: Gesture Pattern Analysis.
With descriptions of the hand shape, movement, and topic of student-produced gestures, all seven interviews were examined regarding patterns of student gesture production. We mapped the gestures produced over the course of the interview to identify emergent patterns of gesture production. Beat gestures, which are simple gestures made without apparent connection to semantic meaning, were also excluded from the mapping (Alibali et al., 2001). Likewise, gesture mapping started with researchers independently mark the topic and time of production of student gestures and followed by consensus coding to reach agreement.
Step 6: Comparison of Student Gestures Before and After Interaction with AR Model.
Lastly, we examined changes in student-produced gestures following engagement with BioChemAR. BioChemAR is an AR based mobile app that allows students to easily load and manipulate a virtual 3D molecular visualizations; previous work has shown that after engaging with an AR model of a potassium channel in BiochemAR, students in undergraduate level biochemistry courses reported increased confidence in their ability to visualize 3D protein structure (Sung et al., 2020). Because BioChemAR introduced 3D models to students, we paid special attention to the differences in the dimensionality of gesturing. Gestures were divided into 2D (defined as being in an xy plane, flat handed) or 3D gestures (defined as being in an xyz plane, typically involving turning, rotation, or some other type of intentional movement in three dimensions). The gestures produced before engagement with BioChemAR were compared with those produced afterwards to inductively code for different types of changes that occurred Table 1. Researchers identified these emergent changes independently first, then discussed to reach consensus on the types of changes that had occurred.
Analysis factor | Description |
---|---|
Dimensionality | Refers to a 2D (in an xy plane, flat handed) or 3D gesture (in an xyz plane, typically involving turning, rotation, or some other type of intentional movement in 3D). |
Spatial relationship | A gesture was counted as exemplifying a spatial relationship if their gestures accompanied verbal descriptions illustrating comparative differences in sizes of/distances between two structures (e.g., “the ions were far apart”). |
Structural detail | Gestures which showed a loose conceptualization of the size, shape, and scale of a K+ channel were counted as lacking structural detail, while those that more precisely defined specific elements of the potassium channel were categorized as having more structural detail |
RESULTS
Our results are organized into three parts. We will start with presenting emergent themes of cospeech gestures produced by students. These findings describe the different themes of information that were expressed through cospeech gestures, as well as the similarity among the hand shapes and movements of gestures that relate to a common theme. In Part II, we expand our analysis of student expression through cospeech gestures to include the temporal dimension and consider additional differences in students’ patterns of gesture production. The introduction of the temporal dimension revealed additional variations in how cospeech gestures may play a role in students’ description of the potassium channel. Lastly, in Part III, we consider the changes in student gesture production following interaction with an AR model of the potassium channel. The differences in how students describe the potassium channel following interaction with an AR model include not only changes in how their verbal expression, but also changes in their cospeech gestures. We will describe three types of changes and illustrate each with an exemplar case. A full set of gestures from our analysis is provided in Supplemental Table S2. A list of participants whose cases were included in our results, including the relevant feature of analysis we highlight in this study, can be found in Table 2.
Participant pseudonym | Appears in | Feature of analysis |
---|---|---|
Jack | Part I | Gestures related to structure, interaction, and comparisons |
Part II | Vertical depictions of ion movement | |
Andrew | Part I | Gestures related to structure, interaction, and comparisons |
Ciara | Part I | Gestures related to interaction |
Part II | Chained gesture production pattern | |
Part III | Precision in depictions of spatial relation | |
Anthony | Part I | Gestures related to relationships |
Part III | Vertical depictions of ion movement | |
Catherine | Part II | Isolated gesture production pattern |
Naomi | Part III | Detail-rich channel depiction |
Part I: Themes of Cospeech Gesture
During interviews, students were tasked with proposing and modifying a model that describes the structure and function of a potassium channel. From categorizing students’ verbal expressions that were accompanied by cospeech gestures, we found that students produced gestures when describing topics related to three main themes: (1) structural components, (2) interactions and movements, and (3) relationships. Each of these themes contains a set of topics that describes components and processes within the potassium channel system (Table 3). These topics provided us with insights about the different aspects of the potassium channel which students used gestures when describing. In our analysis, we found that different students used gestures with similar hand shapes and movements when describing some topics. Below, we describe the illustrative examples of common gestures that emerged from student interviews within each topic. Additional gestures related to each category are in Supplemental Table S2.
Theme | Theme description | Topic | Topic description |
---|---|---|---|
Structural Components | Depictions of components in the potassium channel system and their properties such as size and shape. | Ion depiction | Describes the physical properties of an ion or small molecule present in the potassium channel system (e.g., ion size, shape, or location). |
Channel depiction | Describes the physical properties of the potassium channel (e.g., size, conformation, orientation). | ||
Interaction | Depiction of the movement of a system component or the interaction between two or more components. | Movement through space | Explains some property of movement (typically K+ ion movement) in space, such as speed or directionality. |
Charge-charge interaction | Describes how two or more charged components of the potassium channel system interact with one another (e.g., repulsion and attraction). | ||
Channel-ion interaction | Explains some facet of the interaction between a channel component and an ion traversing the channel. | ||
Comparisons | Adds detail to the description of a structure or interaction by describing differences in magnitude or spatial relationships. | Levels | Describes differences in the magnitude of physical properties (e.g., increasing/decreasing charge, concentration gradient, or energy of a system). |
Spatial relationships | Depicts the arrangement or position of channel components in relation to one another (e.g., distance between an amino acid and K+ ion). |
Theme 1: Structural Components.
When students provided descriptions of the structure of the potassium channel and its associated ions, they often use gestures to add details about the molecular structure such as elements of secondary and tertiary protein structure as well as the general shape of the molecule. Students frequently used gesture to establish the presence of an ion or small molecule in or near the potassium channel (ion depiction). These gestures depicting ions and small molecules are often static, that is, the hand was held in a particular shape without movement. For instance, one student used their fist to depict a water molecule located in the central cavity of the channel (Table 4, Example A). Another student held their thumb and pointer finger in a “pinching” configuration to depict a potassium ion in the channel entrance (Table 4, Example B).
Example | Topic | Gesture name | Transcript | Gesture image |
---|---|---|---|---|
A | Ion depiction | Fist | “There was a lot of emphasis on water being in that central cavity” | ![]() |
B | Pinch | “We don’t want the potassium ions to get stuck in that entrance [of the channel]” | ![]() | |
C | Channel depiction | Lasso | “We see the alpha helices and they eventually taper off” | ![]() |
D | Ring | “the residue lining [of the central cavity] has a certain like diameter” | ![]() |
Students also used gestures to depict larger structural components of the potassium channel. These gestures often involve hand movement instead of static hand shape to provide a representation of some physical characteristics. For example, a gesture that we named “lasso” involves the index finger moving repeatedly in a circular motion. In one instance, a student stated “we see the alpha helices and they eventually taper off” while producing the lasso gesture concurrently with the phrase “alpha helices” (Table 4, Example C). Here, the student used the “lasso” gesture to trace the spiral shape of the alpha helix, adding information about the secondary structure of the potassium channel that cannot be inferred from their utterances alone. In another instance, a student produced a gestural representation of the channel by using their index finger to trace the shape of a circle, providing a depiction of the circumference of the channel’s central cavity (Table 4, Example D).
Theme 2: Interactions and Movements.
When students described their proposed mechanistic model of the potassium channel, they frequently employed movement-based gestures to depict the movements of different molecules and the interactions between them, such as channel-ion interactions and charge–charge interactions.
Gestures that accompanied descriptions of charge–charge interactions between ions, small molecules, and residues within the channel typically relied upon hand motion to depict the dynamic processes at play within the channel. For example, one student made a gesture that we named “pulsing claws” when discussing repulsion between components of the channel. In this gesture, a student’s hands were held in a claw-like formation and rapidly moved back-and-forth from one another, indicating the repulsion between two ions or an ion and channel component (Table 5, Example A). When discussing attraction between a potassium ion and charged residues, students used a gesture that we named “attract,” where one hand was held stationary while the other hand moved towards it (Table 5, Example B). Interestingly, gestures that depicted the same type of interaction may employ different hand shape depending on the objects that were involved in an interaction, while the hand motion stayed the same. For example, when students depicted ions or small molecules interacting with each other, their hands were often held in a fist, mirroring the spherical depiction of ions seen in external representations. However, if the gesture depicted an interaction with a channel component, their hand would often be held vertically and flat, representing the interior of the channel.
Example | Topic | Gesture name | Transcript | Gesture image |
---|---|---|---|---|
A | Charge-charge interaction | Pulsing Claws | “If there were another ion that came into the channel to propel it upward by like that repulsive interaction, um it likely wouldn’t have as strong of an effect” | ![]() |
B | Attract | “We want some attraction of potassium ions, but we don’t want like too much for it to just like get stuck” | ![]() | |
C | Movement through space | Up and down | “Then like the positive charge of the next potassium ion will push it out” | ![]() |
D | Channel-ion interaction | Tube | “It would be like too closed off and another sodium wouldn’t fit in that channel” | ![]() |
In addition to interactions, we also observed cases where gestures were used to depict movements of particles. For example, when describing the movement of ions through space (including in/out of the channel), the “up and down” gesture was used frequently, which consisted of a vertically oriented, top-to-bottom motion of the hand. This gesture was utilized in a variety of contexts and served to simultaneously indicate both motion and directionality of particles. One student used this gesture when describing a proposed mechanism for how a potassium ion might be “pushed” through the channel (Table 5, Example C). In this case, with their palm facing down while moving in the same direction, the gesture depicted the direction of movement of the potassium ions as well as a sense of “pushing”.
While the “up and down” gesture incorporated a sense of “pushing” while depicting the movement of potassium ions, the “tube” gesture instead incorporated structural components of the potassium channel while depicting the movement of ions. The “tube” was produced when the student’s hand was held in a C-shape and repeatedly moved vertically, concurrently depicting the channel and the movement of ions through it. This gesture was used to illustrate why certain ions can pass through the channel while others cannot, and the hand shape was to depict the size of the cavity (Table 5, Example D).
Theme 3: Relationships Between Structural Components or Interactions.
In addition to using gestures to describe the physical objects and processes within a system, students also used gestures to describe the relationships between structures or interactions. These gestures often depicted the quantitative or spatial relationships as they described the potassium channel.
For example, the quantitative relationships between two different states, for example, the relative amount of charge, were often depicted with cospeech gestures. When one student was describing an increase in charge for a positively charged residue, they accompanied their dialogue with a gesture we named “incline”. In this gesture, their hand was angled diagonally and moved upwards (led by their fingers) to describe an increase in charge. The student’s hand moved as if tracing the amount of charge on a graph (Table 6, Example A). Here, rather than depicting the physical existence of a charge (e.g., the “pinch” gesture noted above that depicted the existence of a charged ion), the student used cospeech gesture to depict how the magnitude of charge change over time. Similarly, several students used a gesture that we named “platform shift” to describe energetic changes within the system. The student held their hand horizontally and moved up or down to depict the increase or decrease of energy, respectively. For example, one student used this gesture to accompany their description of the thermodynamic favorability of the presence of a potassium near charged residues in the channel (Table 6, Example B). The “platform shift” gesture depicted the thermodynamic change that occurred within the system.
Example | Topic | Gesture name | Transcript | Gesture image |
---|---|---|---|---|
A | Quantitative Relationships | Incline | “‘Cause then the––the positive charge is just increasing” | ![]() |
B | Platform shift | “A way to bring down the energy to make it like more thermodynamically favorable” | ![]() | |
C | Spatial Relationships | Bracket | “Potassium is [...] the perfect size where that attractive force is like the most effective” | ![]() |
D | Flip pinch | “I would actually flip it, where the top is the intracellular side, and the bottom is the extracellular side” | ![]() |
In addition to quantitative relationships, gestures also depicted spatial relationship between two or more channel components. One student used a “bracket” gesture, in which the thumb and index finger form a bracket shape with the other fingers positioned into a fist shape, while describing the attractive force between the potassium channel and potassium ion (Table 6, Example C). Here, the student depicted the physical distance between structural components with gesture to describe the spatial relationship that made charge–charge interactions within the channel effective in facilitating ion movement. Students also used gestures to depict changes in spatial relationships between channel components. One student utilized a “flip pinch” gesture, in which one hand was held upwards with thumb and index finger in a pinching configuration and quickly rotated. The student made this gesture while stating, “I would actually flip it, where the top is the intracellular side, and the bottom is the extracellular side” (Table 6, Example D). Here, the student made changes to their initially proposed mechanistic model for the potassium channel, and used gesture to describe a shift in spatial relationships.
Part II: Patterns of Production for Different Gesture Themes
In Part I, we described a common set of gestures that were shared by multiple students when describing topics related to the potassium channel. When the temporal dimension was introduced in our analysis of gesture production, we found that students used and/or combined these gestures in different patterns of production. Part I identified the “lexicon” of gestures generated by students in association with different components of their model of potassium channel function; Part II contextualizes how these individual gestures are used in distinct patterns as students formulate their model. In this section, we will describe the different ways in which students used and/or combined these gestures. We found that the patterns of production for cospeech gestures that depicted different themes were also different. We found two distinctive patterns of cospeech gestures: isolated gesture production for gestures that depicted structural components and chained gesture production for gestures that depicted interactions. Isolated gesture production is characterized by producing gestures in much smaller groups over a span of time, in combinations of one or two gestures. Chained gesture production, on the other hand, is characterized by lengthier combinations of gestures over a short span of time (less than 30 s), including upwards of three or more continuous gestures. This context is relevant for adding additional complexity to our description of gesture– for example, students generated a variety of gestures associated with structural components of the potassium channel and with interactions between components. However, these gestures were not all used in the same way– gestures that depicted structural components of the potassium channel were often produced in isolation, while gestures that depicted the interactions between structural components were often produced in chains with other gestures, particularly with gestures that depicted structural components.
To contrast chained and isolated gesture production patterns, we presented below (Figure 1) a visualization of the gesture production patterns during 10-min interview segments where students exemplified isolated (Catherine) or chained (Ciara) gesture patterns. During the selected segments, both participants responded to the question “Could you propose a model of how the K+ ions travel through the channel?” Catherine used fewer gestures and typically used only one gesture at a time. On the other hand, Ciara used multiple gestures in succession. Although these were instances where only one particular production pattern was observed during an interview, it is important to note that other participants exhibited some combination of the two patterns during their interviews.
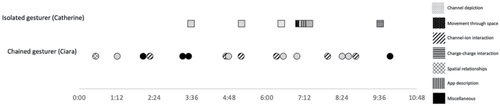
FIGURE 1. Frequency of gestures made during a 10-min period following introduction of BiochemAR app for students exemplifying an isolated (Catherine) or chained (Ciara) gesture pattern. Students used approximately the same amount of time to answer the interview question in this section of analysis. Gesture frequency is plotted as a function of minute of time elapsed during the interview section, with each icon representing a gesture made by the student. The topic of each gesture is indicated by icon pattern (displayed in legend, right).
Isolated Gesture Production for Depicting Structural Components.
Isolated gesture production is characterized by the sporadic creations of visual representations that aligns with spoken words, often depicting structural components of the potassium channel. The interview with Catherine is an illustrative case for isolated gesture production. She seemed to prefer to make only one or two gestures at a time, using gestures sparingly to emphasize certain aspects of her speech. Throughout her 24-min interview, she produced just 30 gestures, a frequency of gesture production that was lower than that of other interview participants. These sporadic gestures appeared to depict structural components of the potassium channel and their physical characteristics. For example, when Catherine described what the potassium channel model looked like, she used isolated gestures to represent different parts of the channel, including the diameter of the selectivity filter (Table 7, Example A), property of particular amino acids lining the channel (Table 7, Example B), and location of the middle of the channel’s inner pore (Table 7, Example C).
Example | Transcript | Gesture image |
---|---|---|
A | “It could only have those interactions if it’s like a certain… diameter” | ![]() |
B | “I would assume that there should be like, what’s it called, aromaticity on the phenylalanine” | ![]() |
C | “Then it gets into the pore part in the middle where it’s––” | ![]() |
Chained Gesture Production for Depicting Interactions and Relationships.
While isolated gesture production exhibited by students like Catherine was often used to depict structural components and add structural details and visual richness of their descriptions, chained gesture production often depicted dynamics of the system in addition to visual details. We define chained gesture production as producing three or more continuous gesture combinations over a short span of time where multiple gestures were linked together one right after the other.
Ciara exemplified chained gesture production during her interview, producing a total of 131 gestures in 49 min. As Ciara described the process by which potassium ions move through the channel, her hands started by making a hollow tube shape that corresponded to the channel, followed by moving her hands up and down vertically to illustrate each of the compartments of the channel. She then held one hand in a tube shape and put the fingers of the other hand into the tube, depicting the movement of potassium ions inside of the channel. She embodied the movement of the ion through the channel by drawing her hand downwards, then moving it back up to start the movement of another potassium ion. These gestures are chained together to create a detailed visual representation to accompany spoken words (Table 8). In this situation, Ciara’s description of the potassium channel involves a series of gestures chained together as a group, rather than isolated gestures that were produced individually.
Timestamp | Transcript | Gesture image |
---|---|---|
19:58 | “you have your channel here but in the channel there’s like a first compartment, a second compartment, a third compartment” | ![]() |
20:06 | “so like it lodges in the first compartment and then another potassium comes and like kind of pushes the first potassium down into second compartment where they’re both stabilized” | ![]() |
20:10 | “like kind of pushes the first potassium down into second compartment where they’re both stabilized” | ![]() |
20:24 | “potassium keeps like pushing the next potassium down and then it gets stabilized um in a new environment until it gets pushed down again by another potassium and then eventually leaves the channel” | ![]() |
In summary, chained gesture production provide additional details about relationships, such as depictions of distances to show spatial relationships, or interactions between the structural components. In contrast to isolated gesture production, chained gesture production often depicted interactions and relationships that described dynamic processes, where a series of gestures showed the changing states of the system.
Part III: Changes in Student Gesture Production Following Interaction with AR Visualization of the Potassium Channel
The interview task was structured where students first proposed a model for the potassium channel without any external representation presented to them, then engaged with an AR visualization of the potassium channel (via BiochemAR), and lastly revised or added additional information to their proposed model based on their interaction with the AR model. In Part I, we categorized the themes of students’ gestures as they described different aspects of potassium channel; in Part II, we highlighted contrasting patterns of gesture production that lent additional complexity to how student descriptions of the potassium channel were conveyed through embodied means such as gestures. In Part III, we will explore how student descriptions of the potassium channel had changed after engagement with an AR model of the potassium channel.
The focus of Part III is to discern the changes in the ways in which students produced gestures when describing the potassium channel following engagement with a 3D AR visualization. Utilizing the topics described in Part I, we analyzed the gestures used for each topic before and after engagement with the AR visualization. We present in the analysis below three types of changes in gesture production that occurred after interaction with the AR model. Broadly, these changes involve the number and types of gestures that were produced when the student is talking about a particular topic. We observed the most prominent changes in gesture production in three topics—ion movement, spatial relationships, and channel depiction. The three cases we will explore in the following sections are summarized in Table 9. Notably, these changes in gesture production were observed when student’s utterances were similar, highlighting that the changes in how students describe protein structure after viewing new external representations are also expressed through gestures.
Case | Gesture shift | Description | Example of gestural shift |
---|---|---|---|
Anthony | Vertical depictions of ion movement | Before using BiochemAR, Anthony primarily uses horizontally oriented gesture to describe ion movement. | Before: Push“[Molecules are] able to push through [...] the bilayer”
![]() |
After interacting with the model,he used more gestures contained in the vertical plane. | After: Up and down“The way this channel’s working is the K+ ions are gonna move, like, down towards the […] towards the QR code.”
![]() | ||
Ciara | Precision in depictions of spatial relationships | Before using BiochemAR, Ciara used gestures that were spatially undefined when describing the positioning of two or more channel components relative one another. | Before: Open Bowl“[…] won’t have two molecules interacting if they’re super far away.”
![]() |
After using the app, she began using gestures that more precisely defined the distance, orientation, and arrangement between structures. | After: Pinch“A sodium ion–I don’t think is big enough.”
![]() | ||
Naomi | Detail-rich channel depiction | Before using BiochemAR, Naomi used gesture that vaguely depicted physical properties of channel. | Before: Funnel“[The ion] would be attracted to the entrance.”
![]() |
After interacting with the AR visualization, she used gestures to illustrate a more detail-rich description of the potassium channel, often highlighting specific features of the channel. | After: Ring“[…] a ring of yellow carbons in kind of like a circular pattern down toward the bottom.”
![]() |
Change in Gestures that Depicted Spatial Orientation.
We observed changes in gestures that students used to accompany explanations relating to ion movement. Prior to seeing the AR model, we observed that Anthony used a wide range of gestures primarily in the horizontal plane, either moving towards/away from the interviewer screen or left/right, to describe ion movement. He used a total of eight different gestures when describing ion movement, the majority of which were either a “push” gesture (wherein one or both hands palms facing toward the screen physically push forward) or “tide” (a gesture similar to the “push” gesture, with an added rounded motion at the beginning of the gesture; Table 9). Anthony used these gestures to imply a direct physical interaction between the potassium channel and passing ions, hypothesizing that molecules will be, “able to push through [...] the bilayer,” or that a chain of interactions between different molecules may be required to finally result in a K+ ion being able to “make it through” a K+ channel.
After interacting with the AR model, Anthony’s gesture production shifted to using more gestures contained in the vertical plane when describing ion movement. As shown in Table 9, most of these gestures were an “up and down” gesture. We want to note that this shift to a vertical plane appeared to incorporate the orientation of the AR model (in which the channel is oriented vertically in a hypothetical horizontal membrane; Figure 2) as a guide to describe the directions and flow of molecules. Anthony stated, “the way this channel’s working is the K+ ions are gonna move, like, down towards the […] towards the QR code,” and, “my guess is though any water molecules would be left at the top. Like left behind.” Moreover, Anthony’s use of vertical movements also seems to suggest a specific endpoint for ion movement in relation to the potassium channel.
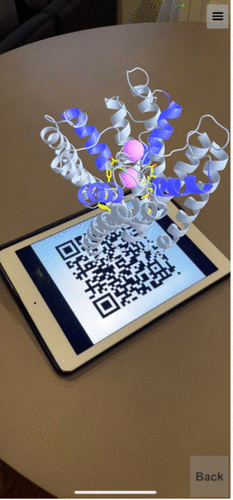
FIGURE 2. Screen capture view of the virtual 3D potassium channel viewed in Model 1 of the BiochemAR mobile app, using a QR code displayed on a tablet.
Anthony’s gestures that depicted the spatial orientation of the potassium channel changed following interaction with the AR visualization. Anthony, like all the other students, interacted with an upright model of a K+ channel on their phone screen. Although all students had the freedom to interact with the model in any way that they wanted, most students chose to move around a stationary model rather than rotating it. Consequently, the model itself consistently remained in a vertical orientation, and Anthony’s gestures that depicted ion movement incorporated the model’s vertical orientation. As Anthony adopted the orientation of the AR visualization, the variety of gestures used by Anthony to describe ion movement also decreased to depict movements only in a vertical orientation. Anthony’s utterances before engagement with the AR visualization did not contain specific information about the orientation of the potassium channel, however, his gestures suggested that the potassium ions were moving along a horizontal line. The change in the spatial orientation of how Anthony was describing the potassium channel was expressed through gestures.
Change in Gestures that Depicted Spatial Relationships.
We also observed changes in gestures that accompanied descriptions relating to spatial relationships. For example, before interacting with the AR model, Ciara produced similar gestures when describing spatial relationships. These gestures were variations of the “open bowl” gesture, where both hands were curved and held slightly away from one another (Table 9). These open bowl gestures were typically used to describe general distances between two structures. For instance, Ciara stated “won’t have two molecules interacting if they’re super far away” while producing the “open bowl” gesture. To emphasize any decrease in distance between two structures, Ciara would use either a “collapse” or “bracket” gesture, representing a shortened distance between her hands or fingers. For instance, she stated “you get a little like even a smallish amount of distance away” while producing the “bracket” gesture. With these gestures, there were no defined distances or stopping points between Ciara’s hands when she described relative sizes or spatial relationships.
After interacting with the AR model, we observed that Ciara shifted to using gestures with more defined handshapes when depicting relative sizes or spatial relationships. For example, Ciara frequently used a “pinch” gesture, consistently leaving approximately an inch of space between her fingers when depicting ions (Table 9). As she was producing the “pinch” gesture, she stated “a sodium ion–I don’t think is big enough,” or, “there’s a little bit of space between two ions”, using the gesture to highlight the ion’s small size in comparison to a potassium channel.
Ciara’s gestures shifted from primarily “open bowl” to primarily “pinches” after interaction with the AR model. Although Ciara’s utterances did not change notably (“a smallish amount of distance” vs. “a little bit of space”), the shift in how spatial relationships were depicted in gesture production highlighted the difference in how Ciara described the various sizes and scales of distances between structural components of the potassium channel. After engaging with the AR model, Ciara was more inclined to use gestures with set distances and placements, typically using the protein model as a guide to mark precise locations, sizes, and movements of structures in space.
Notably, Ciara also interacted with the model as if it were a physical object, often using gestures that directly referenced placements of structures depicted in the AR visualization. After seeing the visualization, Ciara often used the “point” gesture to emphasize the locations of ions. For example, she stated “there’s a potassium like up here and then there’s a potassium here" while pointing at the space where the AR model would have been place if it were a physical object. In this case, Ciara’s utterances of “here” is only meaningful when considered along with the “point” gestures that expressed the spatial relationship between the two potassium ions.
Change in Gestures that Depicted Structural Components.
In our last case, we will discuss observed changes in gestures used by Naomi. When describing the structure of the channel before interacting with the AR model, Naomi primarily used gestures such as “vertical pipes,” where both hands maintained a consistent distance as they descended, and “funnels,” where both hands taper closer together as they descend (Table 9). For example, Naomi produced the “funnel” gesture while stating, “the ion would be attracted to the entrance,” as well as when she was describing where a K+ ion is located, stating, “within the transmembrane protein.” Here, Naomi used the “funnel” gesture for describing the physical structure of the K+ channel, regardless of how general (i.e., referring to the protein as a whole) or specific (i.e., referring to specific residues/domains/structural components) her verbal descriptions were.
After interacting with the AR visualization, Naomi employed a greater variety of gestures when describing the potassium channel. For example, Naomi gestured a “ring” with her finger to describe residues in the entrance of the channel, stating “a ring of yellow carbons in kind of like a circular pattern down toward the bottom” (Table 9). Naomi used a “tube” gesture to describe the central pore of the channel as “sort of like a cylinder-looking barrel going down.” Naomi also continued to use the “funnel” gesture, describing that “the shape of the protein would have to get smaller than the bottom”. After interacting with the AR visualization, Naomi seemed to use additional gestures when depicting the potassium channel, changed from using the “funnel” gesture to depict the entrance, central pore, and the amino acid residues of the potassium channel to using additional gesture such as the “ring” and the “tube” to highlight specific structural components of the potassium channel. Naomi’s gestures that depicted structural components changed to be more diverse and specific, while her utterances remained similar. Attending to Naomi’s gestures showed that students’ description of the potassium channel may become more specific after engagement with an AR visualization, and the increase in specificity of description may be expressed through gestures.
DISCUSSION
The objective of this study was to describe how gestures are incorporated in undergraduate biochemistry students’ expressions about potassium channel structure and function and how students’ gestures changed following engagement with an AR model of the channel on their subsequent gesture production. In our analysis, we described: 1) the themes of students’ cospeech gestures, 2) patterns of production for different gesture themes, and 3) changes in student gesture production following engagement with a 3D AR visualization of the potassium channel via use of BiochemAR.
As described in Part I, we categorized common sets of gestures used by students to describe different aspects of the potassium channel’s structure and function. These gestures may add information to the content of their speech, so attending to student-produced gestures in addition to their utterances can provide a fuller account of how students express their ideas related to biochemistry. For example, students may produce the “pulsing claws” gesture when talking about charge–charge interactions. The produced gesture depicted particles constantly moving closer and further away from each other, expressing information about the relationship between the movement of the particles and charge–charge interactions. As new external representations (in the form of an AR visualization of the channel) were introduced during the interview, attending to gesture production also highlighted additional changes in how students describe the potassium channel. Therefore, student gesture production can be a valuable source of information (in addition to utterances and inscriptions) for studying how students express and communicate ideas related to biochemistry.
Spatial information was a major theme expressed in students’ gestures. Because visuospatial skills and competencies are often associated with the processing of nonverbal information about spatial relationships, attending to gestures can expand analysis of student by considering students’ nonverbal expressions of spatial relationships and complement existing research that often rely on primarily verbal responses.
As described in Part II, we also found that students used two distinct patterns, isolated gesture production and chained gesture production, to produce gestures related to different themes. In addition to the themes of student gestures, these gesture production patterns were also a critical component of characterizing student gesture production and use during these tasks as they introduce a temporal dimension to our description of student gesture production. In our analysis, we observed that these different patterns of gesture production were used for different topics: isolated gesture production often was used when describing channel structure, and chained gesture production when describing dynamic interactions and channel functions. Our analysis resonates with existing studies have used gesture timing and frequency as a salient feature of gesture production in educational spaces (Davis et al., 2021a; Davis et al., 2021b).
Becvar et al. (2008) discussed the use of representational gestures by researchers in biochemistry lab meetings, particularly attending to how these gestures were used to modify and embody materials like diagrams and graphs. We note that we also observed instances of biochemistry students creating and interacting with drawings while producing gestures. For example, when explaining a model for how the K+ ions traverse through the channel, one student, Jack, drew a simple image of a channel on a sticky note and held it up to the camera as he guided his pencil through the channel in order to explain his conception of ion movement. He returned to this drawing several times, using the image as he explained aspects of the channel’s structure which he believed impacted ion movement.
It has been previously determined that gestures could serve as a link between the external representations that students viewed and internal representations that they created for themselves (Srivastava and Ramadas, 2013). In our analysis in Part III, we found that there were changes in student gestures following engagement with an external representation. For example, gestures used by Ciara before she engaged with the AR visualization reflected a uniformity in shape and distance (regardless of the objects in question), with limited variation with respect to biological scales. After engaging with the external representations in BiochemAR, Ciara’s gestures changed to show clear delineations of beginning and end points when considering distance as well as a broader range of gestures that correspond to distances between different types of objects (e.g., between two ions vs. between the ion and the channel). However, future work is necessary to demonstrate how gesture can be used for assessing student internal mental models and/or the impacts of gesture on students’ evolving mental models as they engage with new pedagogical content.
Our observation that undergraduate biochemistry students could produce detail-rich gestures differs from the observation of Myers (2008), who noted that novice crystallographers often had trouble translating on-screen graphics from modeling software like PyMOL into tangible models. This was reflected in their gestures, which were often observed to imprecisely circumscribe an object rather than define its structure with the gestural precision of an expert crystallographer. In contrast, we noted that after using BiochemAR, undergraduate students often produced detailed gestures to describe their model of the channel, mirroring the descriptive gestures produced by the expert crystallographers reported by Myers (2008). This may be indicative of the utility of extended reality (XR) technologies like AR for providing novice biochemists with visualization tools.
Future Directions
Further work beyond the scope of this study is required to more fully characterize the role of gesture in biochemistry teaching and learning. We note that the use of gesture to express scientific ideas is less standardized than the use of language. The conventions of language use established by the scientific community, such as definitions of terms and concepts, guide the use of language in teaching and learning. One important way of identifying student misconceptions relies on identifying the differences between students’ verbal descriptions of scientific concepts and experts’ descriptions. However, gesture production is often fluid and situational, without the same norms that are established by the scientific community. Therefore, the gestures categorized and interpreted in the current study, although they may be commonly shared by students and experts, cannot be compared with established norm to assign the correctness of the gestures. Although there is no “correct” way of using gestures to express scientific concepts, studying gestures can provide valuable insights for understanding the different ways through which scientific concepts are expressed in educational settings.
Moreover, analyzing student gestures in relation to visualization technologies in biochemistry undergraduate education remains an underexplored research topic. In particular, previous visualization software packages largely focused on manipulation of a virtual 3D object within a virtual environment using a mouse or keyboard; newer XR technologies (including AR and virtual reality) afford new ways to interact with virtual 3D visualizations displayed using handsets, physical rotation of QR codes, etc. These new ways of interacting with virtual 3D visualizations could benefit from analyzing gestures as a bridge between 3D visualizations and student expressions. Future work is necessary to understand the impact of these educational technologies on student gesture production.
Lastly, the findings presented in this study only included gesture production in interview settings. Future work is needed to explore student gesture production in biochemistry classroom settings, particularly during group activities where students need to negotiate and coconstruct meaning. Previous work has found that K–12 students working together in groups attend to each other’s gestures and advance the group’s understanding of the subject matter (Singer et al., 2008). Further work can help elucidate the role of gestures in the negotiation of meaning during group activities in biochemistry learning environments.
Previous literature illustrated the importance of instructor gestures on student learning (Alibali et al., 2013; Myers, 2015; Abels, 2016; Yeo et al., 2018). Even without formal training, instructors are able to derive meaning from the gesture of children during problem-solving exercises (Alibali et al., 1997). In our study, we reported changes in student gesture production following engagement with pedagogical material. Therefore, considering student gestures as a form of real-time feedback regarding students’ engagement with the material could naturally complement student-centered pedagogies in undergraduate biochemistry education. Future research can also explore the value of utilizing gesture as a pedagogical feature to engage students.
CONCLUSION
In this study, we examined how students use gestures when describing aspects of the potassium channel and how their gestures change following engagement with a 3D AR visualization of the channel. Our findings provided a detailed description of student gesture production and highlighted that gestures were an integral part of student expressions. This study illustrates the importance of considering both utterances and gestures when trying to understand student expressions of biochemistry content and how these expressions change. Attending to both verbal and nonverbal expressions can help biochemistry educators and education researchers see a fuller picture of student expressions.
ACCESSING MATERIALS
The BiochemAR mobile app is available to download for iOS in the App Store (https://apps.apple.com/ca/app/biochemar/id1434332440) and for Android from Google Play (https://play.google.com/store/apps/details?id=edu.carleton.its.biochemAR&pli=1).
ACKNOWLEDGMENTS
This research was supported by NSF1841992. We thank Jeremy Hsu for helpful feedback on the writing of this manuscript. We would also like to thank Juliana Bachulis for her willingness to be a photographic model for the images shown throughout the manuscript.